Physics is the science of understanding Nature through mathematical formulations. Here understanding amounts to make a model within a given theoretical framework, analyzing the model to make predictions and performing observations or experiments of natural phenomena to check the predictions. Once predictions of a model pass this test, physicists claim to understand the phenomenon. This notion of science and in particular physics was mainly initiated by Galileo Galilei and is continued to date. Newton was the first to formulate mechanics of bodies and objects. According to Newtonian dynamics, we have a notion of force which quantifies how particles and systems interact and see each other. In this setting, therefore, one in principle knows everything about the dynamics of a system once one can formulate the forces acting on the system and its constituents. Since the time of Newton, physicists have tried to formulate forces, or in modern terminology interactions.
Gravity and Einstein General Relativity
Gravity is the force of Nature historically formulated first. Nevertheless, it still remains very much challenging to date. Newtonian gravity[1] is the force which is exerted on any mass, like us, the Earth, the Sun or any other object, and is proportional to the inverse-square of the distance between the two bodies. Gravity is a universal force which seems to be present everywhere and among all physical objects. Despite the successes of the inverse-square law of gravity in explaining dynamics of objects on Earth and celestial bodies, like planets of the solar system, it was challenged, to be more precise, improved and corrected, over two centuries later by Einstein’s theory of General Relativity (GR). Einstein’s relativity states that time and space are closely related concepts and it is hence more fruitful to treat them on the same footing, and use the notion of space-time rather than time and space individually. According to the Einstein GR a force as universal as gravity should be linked with something as fundamental and universal, that is the space-time itself. In Einstein GR, gravity is tied with a property of space-time called metric which is a measure of the distances in space-time and strength of gravity is measured by another property of space-time called curvature; the bigger the curvature, the stronger gravitational pull one would feel. Here curvature has essentially the same meaning as one intuitively understands about curvature in surfaces we deal with in daily life. However, instead of two dimensional surfaces, here we have four dimensional space-times.
Einstein GR was introduced in 1915 and like all the other physical theories or models it is described by an equation. Einstein’s equation takes the matter and energy distribution as an input and its solutions determine the space-time which is caused as a result of the gravitational pull of the matter and energy inside it. Einstein equations are nonlinear coupled partial differential equations and no general method to solve them exists to date. Nonetheless, from the early days these equations were introduced, physicists and mathematicians alike, have been working hard to find solutions to these equations. Among the first solutions were the one introduced in 1915 by German physicist Karl Schwarzchild, and the one given by Russian mathematician and physicist Alexander Alexandrovich Friedmann in 1922. The corrections introduced by Einstein GR to Newtonian gravity are generically tiny in scales we deal with in our daily life, while they are more pronounced in larger scales, or extreme conditions of very high matter density or temperature, or very short, sub-atomic distances which we have not been able to probe so far. So, GR may be put to test in some different fronts and distance scales, cosmic scales (distance as large as galaxy cluster size or bigger) where a Friedmann-type solution is expected to be at work; astrophysical scales, the Solar system physics and eventually very-very short distances.
The first success of Einstein GR was made through an analysis by Einstein himself. Considering the corrections GR provides to Newtonian gravity, it was shown that GR, and in particular the Schwarzchild solution, is capable of explaining the mismatch of observations accumulated for a couple of centuries to early 1910s about the orbit of Mercury with the predictions of Newtonian gravity. However, the first experiment designed to test GR came in 1919 and was based on Schwarzchild’s solution and the so-called light-bending or more technically as it is known today, gravitational lensing, the fact that within GR setup light will bend while passing around a massive object, like the Sun. Later on some other solutions of GR (other than Schwarzchild) passed observational tests, and to date GR has appeared very successful in confronting observational data.
Black holes and horizons
Schwarzchild’s solution has some peculiar features and theoretical issues which in particular made Einstein very unsatisfied and unhappy: the solution has a singularity in some specific locus of space-time, where gravity is very strong and curvature blows up. In addition and as pointed out, presence of gravity generically changes the causal structure of space-time and how light rays travel in the space-time. In particular, this causal structure may be distorted to the extent that there exists a region of space-time which only allows for one-sided communications: one may be able to receive signals in that region but not send signals out. The border beyond which one can’t make two-sided communications is called horizon. Fortunately, in the Schwarzchild case and presumably in all physically relevant cases, the singular region is dressed and is not naked; it is hidden behind the horizon. In other words, singularity is inaccessible to anybody outside. This may make presence of a singularity more tolerable.
John Wheeler coined the name black hole in 1967 for space-times that have horizons with observers living outside the horizon. Schwarzchild’s solution is the simplest black hole predicted by the GR theory. Black holes, as predicted by the theory, are indeed very odd objects: if one approaches the horizon one may simply pass through without feeling anything special, however, once one passed it, there is no escape and no return. Distortion of causal structure of the space-time are such that it will take infinite time, no matter how hard you try, to go back across the horizon after you crossed it once. Moreover, according to the theory, once one passed the horizon the destined fate is falling into the singularity. Close to the singularity gravity effects and tidal forces are so strong that one would be torn apart, not of course a pleasant fate. So, the key question is whether black holes are just theoretical fantasies or they can actually form from ordinary matter we find in the world? Another key question is whether such a solution of the GR theory can actually exist in the real world? These two questions have both theoretical and observational aspects, which we discuss next.
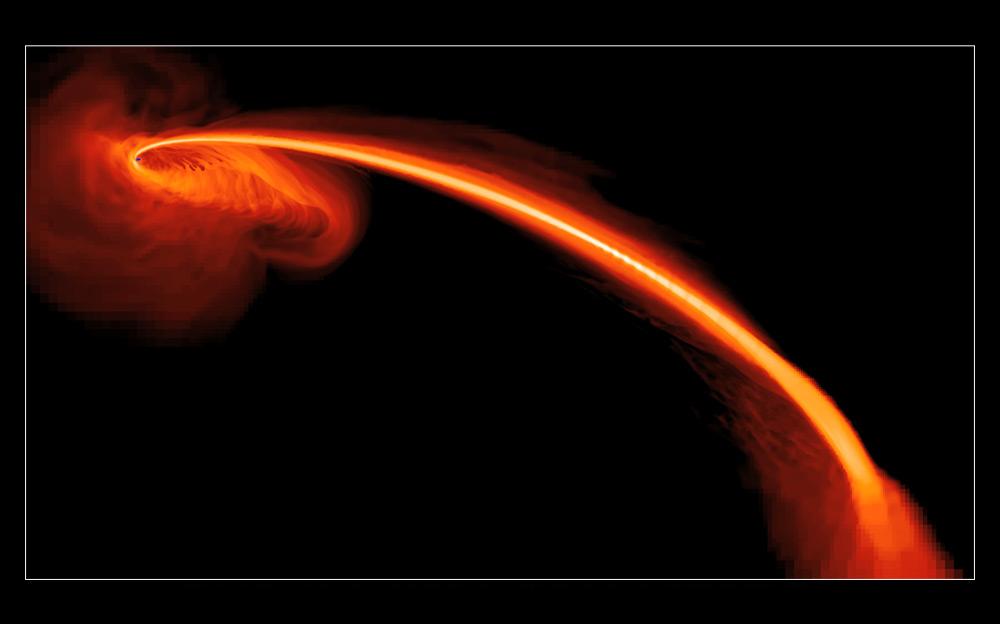
Where do black holes come from?
Recall that source of gravity is the mass and as we increase the mass the gravity pull increases. Using naïve intuition coming from Newton’s inverse-square law, increasing mass its gravitational pull can compress it to a region smaller than the corresponding horizon size. The question is whether in reality such a squeezing can take place. One may make a thought-experiment. Let us consider a lump of gas at low temperature and leave it for a long time. This is in fact very close to the situation almost all stars we see today have started from. Under gravitational pull, the molecules of the gas start condensing and squeezing in. If there is no other force acting on these molecules, there is in principle nothing to forbid or stop the squeezing process and the system undergoes the gravitational collapse and a black hole forms.
In reality, however, there are other forces. These forces set in when the matter is squeezed to the extent that individual atoms begin to get pushed and squeezed into one another. So, the internal structure of atoms and in fact even the atomic nuclei also become important. This makes the nuclear forces become important much before the black hole can form. This is the simplest model for the star evolution: when a lump of gas squeezes in under its own gravity it reaches densities and temperatures where nuclear fusion can start and it becomes a star like our own Sun. This leads to repulsive forces which are generically strong enough to withstand gravitational collapse. The evolution path a star follows very much depends on its mass and one should hence modify or improve the above simple minded picture. There is a critical mass above which these other forces, which are of genuine quantum nature, cannot withstand the collapse. This critical mass is about 8-9 times the mass of our own Sun, see chapter 1 of [1] and references therein. That is, starting with a heavy star, at the last stages of its evolution it undergoes the dramatic supernova explosion and what remains after the explosion is a black hole.
The above core-collapse scenario leads to formation of stellar mass black holes with masses 1-10 times mass of our Sun. However, theoretically, black holes can exist in a very wide range of masses, from microscopic black holes (as light as 10^(-8) kg) all the way to supermassive black holes (as heavy as a typical galaxy like the Milky way). These black holes are not formed through the core-collapse scenario. Some of them can be primordial black holes, remnants from the early Universe. Some heavier ones can be a result of black hole mergers which happen in the late universe and within galaxies. As yet the origin of supermassive black holes remains an open question.
Black holes in the sky?
Existence and physical properties of black holes, depending on their mass, can be checked either in terrestrial experiments or astrophysical observations. On the other hand, we can see things only when a light (or some other signal or messenger) can come out of it and reach us. But, we just argued that no emission comes out of black holes, so how can we test this prediction?
The answer can come from the fact that black holes affect their surroundings. One proposal is that black hole can eat up and absorb in stuff: Before falling into the black hole and passing the no-return point, matter leaves behind a trace; the matter accreting into the hole speeds up, heats up and starts radiating off X-ray or more energetic γ-rays with specific characteristics and this radiation may be observed. Another proposal to detect black holes is through the light-bending and gravitational lensing the black hole may cause when the light or a generic electromagnetic wave from other sources pass in vicinity of a black hole. In the last decade another window to observing black holes has opened, observing them through gravitational waves emitted if we have merger processes which involve black holes.
The emerging picture is that at the center of a galaxy we have a (super)massive black hole and the whole galaxy is rotating around this black hole whose mass can be as large as the mass of the rest of the objects in the same galaxy. Moreover, we now know that black holes of masses few to 200 mass of the Sun are very frequent and all over the place [2]. Multi-messenger astrophysics will continue to provide a lot of more information about black holes in the sky, their origins, formation and evolution in the years to come.
Black holes, Quantum theory and information problem
Physics of black holes still holds a lot of mysteries and many physicists are working hard to open up this black box and uncover its mysteries. One of these mysteries on the theoretical side arose from seminal works of Stephen Hawking since the early 1970s, indicating that black holes are not completely black and actually radiate (known as Hawking radiation), once we combine Einstein’s GR with laws of quantum theory. Hawking radiation can lead to a total evaporation of a black hole, leaving one with an almost blackbody radiation. The process of formation and evaporation of black holes leads to the information problem: all different kinds of matter undergoing gravitational collapse yield the same aftermass, after the black hole has evaporated. This has brought many more questions and mysteries about black holes which are actively under study by theoretical high energy physicists. Black hole information problem has led to ideas that quantum information theory and its concepts are a very natural framework to (re)formulate space-time and gravity [1,3]. We hope these studies bear fruit and have a more coherent and clear picture of black holes, their formation and evolution, sometime soon.
by M.M. Sheikh-Jabbari, School of Physics, Institute for research in fundamental science (IPM), Tehran, Iran
[1] It seems that Hooke, Wren and Halley, who were contemporaries of Newton, had independently discovered this law, but it is largely known as Newtonian gravity.
References:
[1] D. Grumiller and M.M. Sheikh-Jabbari, Black Hole Physics: from collapse to evaporation, Springer graduate textbooks, 2022, ISBN 978-3-031-10342-1, doi:10.1007/978-3-031-10343-8
[3] J. Maldacena, Black holes and quantum information, Nature Rev. Phys.2 (2020) no.3, 123-125